Graduate Fields
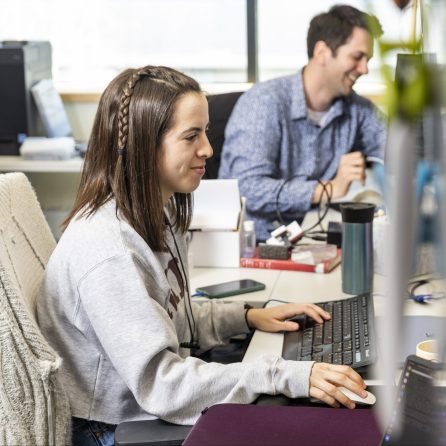
The graduate program in AeroAstro provides educational opportunities in a wide variety of aerospace-related topics through academic subjects and research. Our graduate program includes graduate-level subjects in Course 16 and others at MIT, and research work culminating in a thesis. Degrees are awarded at the masters and doctoral levels.
Fields of Study
AeroAstro offers 13 fields of study for masters and doctoral level students to select based on their desired expertise. Doctoral students must complete a course-based evaluation in their chosen field of study. Students will work with their advisors and/or doctoral committees to determine the sequence in which subjects are taken In addition to these recommendations, the official S.M. and doctoral degree completion requirements must be taken into account during the design of a graduate program. The chosen area from the list below will be noted as the field in which doctoral students receive their degree on the diploma conferred upon them at Commencement.
Intensive computation for simulation and optimization has become an essential activity in the design and operation of complex systems in engineering. While computational science is a discipline in itself, it serves to advance all of science and engineering. Despite the already considerable level of development, it is expected that the next decades will experience explosive growth in the demand for accurate and reliable numerical simulation and optimization of complex systems. It is important to realize the difference between computer science and computational science, the former referring to the science and technology pertinent to the computer, whereas the latter addresses the development of modeling and optimization technology and software for specific systems applications.
Since the early days of computational mechanics, the aerospace community has been at the forefront of computational science and engineering-driven by a need for high accuracy models in the design of aerospace vehicles and systems. Not surprisingly, NASA has played a major role in the development of the early finite element codes for structural mechanics (e.g. NASTRAN) as well in the development of Computational Fluid Dynamics. Traditionally, the aerospace industry with companies such as Boeing. Lockheed, Rolls Royce, Dassault, etc. have pioneered the use of the latest computational tools and in many cases, they have developed highly sophisticated in-house capabilities through alliances with universities and research institutions. The origin of the more recent paradigms on multidisciplinary design and optimization (MDO) can also be traced back to the same community. The need for accurate and reliable prediction tools for complex engineering systems will continue within aerospace.
The department has had a strong presence in computational engineering, in particular, but not exclusively in the areas of computational fluid dynamics and computational mechanics of materials, uncertainty quantification, and optimization. Currently, our department has several faculty and staff focused principally on research in computational engineering. These faculty are affiliated with the MIT Center for Computational Science & Engineering which is housed in the newly created Schwarzman School of Computing.
Educational Goals
Simply stated, at the SM level the ACE focus area aims to educate the engineers and scientists in advance computational methods who will computationally model, optimize, control, and operate the important aerospace engineering systems of the next decade, as well as contribute to our own increasingly computationally intensive research programs within the Department. The PhD in ACE program allows students to further specialize at the doctoral level in a computation-related field of their choice through focused coursework and a doctoral thesis. The graduate program is designed with a common core that serves all disciplines, and an elective component to concentrate on particular applications. The educational program is designed to emphasize: breadth through introductory courses in the areas of numerical linear algebra, discretization of partial differential equations and optimization methods; depth in the areas of materials and structures, fluid mechanics, propulsion and control; integration and multidisciplinary aspects; hands-on experience through a research-based thesis.
A defining challenge for aerospace in the 21st century is understanding and reducing air travel’s environmental impacts. With air travel demand forecast to double or triple by mid-century, meeting this will require major advances in aerospace vehicle technologies, changes to the air transportation system, reimagining the energy sources and carriers used in aviation, and reshaping of our regulatory and policy frameworks. For example, if aviation is to halve its climate impacts by mid-century while growing at projected rates, there will need to be an approximately 80% reduction in the climate intensity of flying.
Aviation is a unique sector in terms of its environmental challenges because it is the only human-made source of pollution emitted at altitude. These emissions give rise to phenomena that are currently thought to double (or more) their climate impact relative to the same emissions at ground level. To know what changes to make to the aviation system, we need to better understand how aviation impacts the environment. This applies not only for aircraft that are in or entering service but for potential new aircraft – perhaps even supersonic aircraft at high altitudes that may give rise to stratospheric ozone depletion. Beyond commercial aviation, the growth in satellite and manned launches also raises the potential for environmental challenges associated with rocket emissions.
The aviation sector is a significant and growing user of oil, and there are energy security challenges as well as the environmental consequences of using fossil fuels. Biofuels are a potential way to address both these issues, but it is far from clear what would be the overall impact of this transition. Beyond fuels that serve as a drop-in replacement for jet fuel, there are other potential energy carriers – cryogenic fuels such as liquefied natural gas or hydrogen, or even electrochemical storage (batteries). Such alternatives have major barriers to overcome if they are to become viable.
Aerospace technologies offer great potential for understanding and protecting our environment. Space-based Earth observation is important for measuring our changing climate and aviation’s impact on it. The rise of small satellites enables new capabilities in distributed environmental monitoring from space, and drone-based environmental sensing combined with advanced atmospheric modeling offers new ways to manage air pollution, monitor emissions, and observe our planet’s ecosystem. Finally, the potential to use aerospace technologies to engineer the earth system to offset adverse effects of climate change – known as geoengineering or climate engineering – may be the ultimate backup plan if global measures to curb carbon dioxide emissions are not successful. But the potential for unintended consequences at a large scale means that the risks need careful evaluation.
With an education in Aerospace, Energy, and the Environment you will be prepared to tackle major multidisciplinary energy and environmental problems facing aviation and to seek opportunities for aerospace to be part of the solution to environmental problems. MIT offers a wide range of subjects related to the topic, as well as a core subject that educates aerospace engineers in the relevant environmental and energy science.
Educational goals
The goal of the Aerospace, Energy, and the Environment field is to prepare students to address the major environmental and energy challenges facing aerospace. Specific objectives will be decided between advisor and advisee, but a typical graduate of the program will aim to have:
- A foundational understanding of the relevant environmental and energy science as it relates to aviation, including climate science, air pollution, fuels, combustion, energy conversion in gas turbines and other propulsion devices, and alternative fuels and energy carriers;
- A foundational understanding of the air transportation system, aircraft design, and aerospace propulsion as it relates to energy use and environmental impacts;
- An awareness of the interaction of energy and environmental policy with aerospace; and
- A more developed (deeper) understanding of an area in technology, operations, fuels, or policy that can provide the capability to mitigate the environmental impacts of aviation, space launches, or an area in which aerospace technologies contribute to environmental solutions.
In contrast to a rocket engine which, in addition to the fuel, carries along with an oxidizer, an air-breathing propulsion system uses atmospheric air to oxidize the liquid fuel. Air-breathing propulsion systems include the jet engine, the ramjet, and the scramjet. The field of air-breathing propulsion involves various disciplines in science and engineering such as fluid dynamics, turbomachinery aerodynamics, thermodynamics, and materials and structures.
At MIT, research and teaching in this field are conducted at the Gas Turbine Laboratory (GTL) which has had a worldwide reputation for research and teaching at the forefront of gas turbine technology for over 50 years. The concept of an MIT Gas Turbine Laboratory was formulated not long after the first jet engines were successfully run. Shortly after the end of the Second World War, Professor J.C. Hunsaker, who was one of the pioneers of aviation in this country and who was a member of the original National Advisory Committee on Aeronautics (the forerunner of NASA), brought together a group of American industries who donated funds for the construction of a laboratory devoted to jet propulsion. A plaque commemorating the 1947 dedication now hangs in the main laboratory.
The research at the GTL is focused on advanced propulsion systems and turbomachinery with activities in the computational, theoretical and experimental study of (1) loss mechanisms and unsteady flows in turbomachines, (2) compression system stability and active control, (3) heat transfer in turbine blading, (4) gas turbine engine noise reduction and aero-acoustics, (5) pollutant emissions and community noise, and (5) MEMS based high-power density engines.
Examples of past research activities include the first implementation of a three-dimensional computation of the flow in a transonic compressor and the concept of blow-down testing of transonic compressors and turbines (thereby enabling these machines to be used for university scale experiments). Current examples are the work on “smart engines”, in particular active control of turbomachine instabilities, the research project on “micro engines” which involves extensive collaboration with the Department of Electrical Engineering and Computer Science, and the “Silent Aircraft Initiative” which is a collaborative project with Cambridge University, Boeing, Rolls Royce, and other industrial partners, to dramatically reduce aircraft noise below the background noise level in a well-populated area.
The Gas Turbine Laboratory maintains strong ties with industry and government research in the area of propulsion and turbomachinery technology, as well as with other academic institutions that are leaders in this field. For example, there are collaborative projects with the gas turbine engine manufacturers connecting the work in the GTL with “real world” problems, and there are longstanding collaborative efforts with Caltech and with the Whittle Laboratory at Cambridge University in England. From an educational perspective, the close links to the outside world enable the exchange of knowledge and ideas and give the students an opportunity to present work directly to outside sponsors, to conferences, or to other individuals from academia, industry, or government.
Educational Goals
The proposed course of study is intended to introduce and expose the student to the latest technologies and techniques used in the development of the current and next-generation air-breathing propulsion systems. The educational goal of the Master’s program in air-breathing propulsion is to provide students with the foundational understanding of fluid mechanic principles and concepts that are required to enable physical insight into the behavior of a broad class of propulsion devices and to model and solve industrial-strength fluid mechanics problems in a rigorous manner. The successful graduate of the program will have:
- Acquired the fundamentals in internal flow, turbomachinery aerodynamics, and air-breathing propulsion system design
- Developed physical insight into the phenomena which characterize the fluid dynamic behavior of air-breathing propulsion systems
- Acquired the ability to rigorously define the levels of modeling required for a useful description of a number of air-breathing propulsion situations
- Acquired the ability to interpret numerical simulations and experimental results in terms of first
- Generated research contributions to the current air-breathing propulsion body of knowledge
Since Charles Lindbergh’s first solo non-stop flight across the Atlantic Ocean in 1927 air transportation has become a major driver for global travel and commerce. Initially, major cities were connected by only a few pioneering airlines and the focus was on transportation of passengers and airmail. Gradually, with the advent of the jet aircraft, specifically the Boeing 707, air transportation has expanded at a dramatic pace.
The sustained growth of air transportation since the 1960s has led to new generations of wide and narrow-body aircraft, and the emergence of new market segments such as business aircraft for corporate travel. The air shipment of parcels (e.g. DHL, FedEx, UPS, and other carriers) has increased enormously in recent years and is a major enabler of decentralized supply chains and commerce.
With this expansion, however, have come a number of systems engineering challenges, all requiring further research and development of advanced methods:
Capacity Constraints: As the number of commercial flights increases along with the number of passengers carried per year, a number of capacity constraints are affecting the efficiency of the air transportation system, as well as its ability to expand further. The two main constraints are airport runway constraints (the number of takeoffs and landings that can be performed per hour), as well as en route air traffic control constraints. Air transportation experts and researchers are investigating the effects of these capacity constraints as well as potential ways of removing bottlenecks from the air transportation system, either through improved infrastructure and technology or by the adoption of new procedures.
Safety and Security: The terrorist attacks of September 11, 2001, have demonstrated in a tragic way that large commercial aircraft are vulnerable to targeted attack and that such vulnerabilities must be mitigated through improved security at airports, in aircraft themselves, as well as by developing best practices. Additionally, while the number of aircraft accidents and fatalities has decreased significantly since the 1960s further improvements in air safety need to be achieved in light of the growth in air traffic volume and density.
Environmental Impacts of Aviation: Airports around the world, led by Heathrow in London (UK) and several other Western European airports, are imposing increasingly stringent regulations to limit the impact of noise, NOx, and particulate emissions on the local population surrounding airports. One way to reduce the impact of aviation is to develop a new generation of more environmentally friendly aircraft.
As urban areas expand, airports that used to be well outside city limits are increasingly surrounded by urban areas. This requires the careful development of new approach procedures that will minimize the noise footprint of aviation in urban areas. It also requires a more integrated approach to airport planning and management and a more deliberate and strategic approach to stakeholder interactions. There is also a desire to understand environmental impacts at a global scale and develop phenomenological and parametric models of air transportation at such scales. This is currently one of the aims of the PARTNER consortium, in which MIT is the leading institution.
Economics and Evolution of Airlines: Since the airline deregulation of 1978, U.S. airlines have faced many challenges and experienced great year-to-year variability in terms of profitability and industry health. This has manifested itself through mergers and consolidation and through the disappearance of some of the major airlines of the past (PanAm, TWA, Eastern). While some of the traditional airlines are struggling, newer low-cost airlines (Southwest Airlines, Jet Blue) have made significant inroads in restructuring the industry and challenging traditional business models. A thorough understanding of airline economics and of interactions with government regulations, market demand, and technology is required to make contributions in this important area.
One of the challenges facing air transportation systems engineers is that they must understand the interaction of a number of complex, interrelated domains. This interdisciplinary focus is essential to future success as a researcher or practitioner in this area. Below we list what we feel are the main disciplines that are necessary to master air transportation as a field. Different individuals will choose to place special emphasis on one or two of these areas, but some familiarity with all of them is necessary.
First, they must understand aircraft technology and design with an eye towards operational issues. Each type of aircraft is characterized by a set of specifications such as range, capacity, and takeoff and landing length. These specifications drive the way in which aircraft are used to transport passengers and/or cargo as part of a larger transportation network. The aircraft also consume fuel and produce noise and chemical emissions. For the most part students in Air Transportation will not focus directly on the design of aircraft, but rather on the implications of the aircraft’s technical, economic, and operational characteristics in a larger context.
Infrastructure aspects are also crucial since they relate to the abovementioned capacity constraints. The planning and design of new airports are major technical, economic, and policy challenges. Airport runway and taxiway layout, optimization of approach and departure procedures, and integration with the local economy and ground transportation systems must all be considered. The air traffic control system (ATC) is also a major part of the infrastructure. Radar systems and navigational aids (such as GPL, VOR, NDB, etc.) have been used for decades, but their modernization and upgrading have lagged behind the best available technology due largely to policy and economic challenges. How can a major infrastructure be upgraded, when its day-to-day operations cannot be disrupted? These are major questions needing to be addressed by air transportation engineers.
Knowledge in operations research, economics, and policy is essential for tackling major air transportation questions. Airlines typically operate as for-profit firms providing transportation services to passengers and cargo. The modeling of such operations, including the purchase and leasing of new and existing aircraft, the scheduling of aircraft and crews, the monitoring of direct operating costs, as well as the susceptibility to fluctuating fuel prices and government regulations are all crucial aspects with which air transportation professionals have to grapple.
Educational Goals
The overall educational goal of the MIT graduate program in Air Transportation is to prepare students for successful careers as researchers and practitioners in the areas of airline management, air transportation infrastructure design and analysis, as well as air transportation systems architecting.
Successful graduates of the program will have achieved the following objectives:
- They will have gained a fundamental understanding of aircraft technology and design, typically focusing on wide and narrow-body commercial aircraft.
- They will have developed the ability to assess emerging trends, such as the growing use of business jets and the fractional ownership of aircraft.
- They will have exercised this knowledge in the analysis and assessment of a particular air transportation problem, using real world data and interactions with actual stakeholders (airlines, FAA, general aviation, airport operators).
- They will have established breadth in the main areas underpinning air transportation: aircraft and airport design, network modeling, computer simulation, airline economics and management.
- They will have gained experience in the field by applying this knowledge in the context of an actual airline or government organization, e.g., through internships or research interactions.
- They will have generated original research contributions to augment the existing body of knowledge.
Aircraft, both manned and unmanned, are an indispensable part of the fabric of our lives, and fulfill a number of roles from transporting passengers and/or cargo, to surveillance, firefighting, and a number of military and other roles. Some major challenges in present and future aircraft design are:
- Dramatic reduction of fuel consumption, noise, and exhaust emissions of transport aircraft
- Design of aircraft with new power architectures such as all-electric or hybrid-electric
- Unmanned Air Vehicle design and operations, including coordination of large numbers of UAVs for operation in open, crowded, or hostile environments
- Design of low cost, safe, and increasingly capable aircraft such as those for Urban Air Mobility
- Designing military aircraft (transport, tankers, fighters…) for increasingly uncertain future operations
Aircraft are extremely complex products comprised of many subsystems, components, and parts. They also operate within larger global air transportation or defense systems. The conception, design, production, operation, and maintenance of aircraft are therefore influenced by many factors including technical, economic, political, organizational, financial, and regulatory.
The engineering of aircraft as a system requires methods, tools, and processes which can successfully address these many complexities. Systems Engineering and Systems Architecting are the fundamental disciplines embodying these methods, tools, and processes. Systems Architecture addresses the overall strategy for developing system-level requirements which meet users’ needs, meet investors’ expectations, incorporate knowledge from past experience, and satisfy regulatory and other constraints. Systems Engineering on the other hand is the process used to develop integrated physical or software components such that the resulting system or product meets the system-level requirements.
The general techniques of Systems Engineering and Architecting techniques can be applied to any of the following system levels:
- Level 1: The air transportation system (aircraft, airports, air traffic management) or the air defense system (aircraft, satellites, missiles, ground stations)
- Level 2: The aircraft and/or related systems (trainers, manufacturing systems, maintenance systems)
- Level 3: Major aircraft subsystems or subassemblies (flight controls, propulsion, hydraulics)
- Level 4: Components (instruments, actuators, pumps)
- Level 5: Parts (fittings, fasteners, blades)
The focus of Aircraft Systems Engineering is primarily on Level 2 – the entire aircraft. Level 1 is addressed in our department’s Air Transportation Systems program, while Level 3 is the focus of many of our other engineering discipline-oriented programs. Although presented as a clean hierarchy herein for the sake of brevity and clarity, it is often the interactions of the various (sub)-systems and/or levels that introduces complexity and a set of key issues that the systems engineer must examine. The systems engineer must, at least, be aware of the levels immediately above and below the levels in which they work and consider the interactions caused by their work within their specific level of consideration.
Educational Goals
The overall educational goal of the MIT Program in Aircraft Systems Engineering is to provide students with a foundational understanding of the systems engineering/architecture process and methodologies required to provide integrated product solutions to technical, economic, and societal needs and requirements.
Successful graduates of the program will have:
- gained a fundamental understanding of systems engineering and architecture.
- developed a working knowledge base of internal and external factors related to integrating an aircraft as a system.
- acquired relevant experience applying systems engineering concepts, processes and methodologies in an aircraft context.
- generated research contributions to the current aircraft systems engineering body of knowledge.
The field of Autonomy focuses on developing embodied intelligent systems, ranging from autonomous drones to self-driving cars and robots, that can physically operate in complex environments with minimal human supervision. Therefore, it includes foundational disciplines including planning and decision-making, control and estimation, sensor fusion and perception, and human-robot interaction, among others. Ongoing research in autonomy aims at designing algorithms and systems that are able
- to perceive and build their own models of the world around them,
- to use those models for making complex decisions over long time and length scales,
- to maintain those models as the systems move around and as the world changes,
- to act in ways that are trustworthy and reliable when deployed in safety-critical and high-integrity applications,
- to enable more effective human-robot teaming.
The goal of this research is to build systems that can assist with dangerous or repetitive tasks, explore remote environments and benefit society.
Educational Goals
A student completing a degree in the area of autonomy will obtain a solid knowledge of planning, decision making, estimation, and inference. In addition, a student will obtain knowledge in a number of closely related fields, such as control, perception, or human-robot interaction. The specific educational plan would depend on the student’s research interests. To achieve this goal each student should develop an educational plan in discussion with their academic advisor.
The increasing reliance on information technology in aerospace makes communications a critical element of most modern space, air, and ground operations. In many scenarios, communication resources and networks are essential for delivering critical information. Both terrestrial and satellite-based networks are needed for providing modern communication-intensive and information-rich services. Department faculty are engaged in a wide range of research activities in the communications and networks area, including wireless and sensor networks, space networks, satellite and hybrid communication networks, location-aware networks, and ultra-wideband systems.
Educational Goals
A student completing a degree in the area of communications and networks will obtain solid knowledge of distributed sensing and communication networks. In addition, a student will obtain knowledge in a number of closely related fields, such as information theory, optimization, control, as well as statistical inference. The specific educational plan would depend on the student’s research interests.
Controls focus on the algorithms and techniques needed to achieve the desired performance in complex dynamic systems. It includes estimation, filtering, and navigation to determine how the system is behaving, and the design of feedback algorithms (classical, optimal, robust, and adaptive) to modify that behavior. In addition to these rigorous analyzes, our focus is on developing practical control systems for aircraft, UAVs, cars, and robots. Current research is addressing the integration of these traditional control approaches with machine/reinforcement learning for real-world systems.
Educational Goals
The educational goals of this field are to develop the algorithmic and software skills to design classical, state-space, and optimal controllers/estimators for dynamic systems. We also focus on the interpretation of how these algorithms achieve the desired performance goals, which is cemented in a firm understanding of the dynamics of the system. The limitations embedded in the assumptions of the approaches is also discussed to highlight the practicality of the techniques. Hardware experiments provide experience in the implementation issues that often arise in real-world systems.
Aerospace systems today can be viewed as complex socio-technical systems. Examples of such systems are the new generation of federated satellite constellations or satellites and high altitude drones working together as a federated system of systems. Many of the significant problems in producing effective aerospace systems today lie beyond the traditional aerospace disciplines (such as structures, aerodynamics, and controls) and encompass new ways to create and operate these systems to achieve the overall goals throughout the entire system lifecycle, from early design activities to deployment and during the operational lifetime. Some of the most significant aerospace engineering challenges lie in creating new frameworks and approaches to decision-making at the larger, integrated system level and not just at the component level or at the individual vehicle level.
Systems today may have millions of parts, all of which must work together to achieve the overall system goals while satisfying constraints (such as safety and security) on how those goals can be achieved. In such systems, early design decisions have a disproportionate impact on
- System performance
- Lifecycle cost
- Lifecycle properties (e.g. the flexibility of a system to perform other missions or to perform their original mission as the system and the environment evolve and change around it)
- Stakeholder value delivered
There is a need for formal methods and tools to assist in making these decisions. Examples of formal methods in Engineering Systems are architectural decision graphs, safety control structures, agent-based modeling, or multi-objective systems optimization to find the Pareto front of non-dominated alternatives.
In addition, seeking more performance and resilience at a lower cost is leading to increasing levels of complexity due to:
- Infusion of new technologies
- Shifting from monolithic to more distributed systems
- Trading of multiple competing objectives (performance, cost, energy) against each other
To make progress, we need to better understand the impact of complexity on engineering. The overall goal of Engineering Systems is to determine how to create more effective sociotechnical systems. How to design and operate such systems is an important current topic that will only grow in the future.
Engineering Systems students study the underlying principles and methods for designing complex socio-technical systems that involve a mix of architecture, technologies, organizations, policy issues, and complex networked operations. The focus is on aerospace and other complex systems critical to society. A unique emphasis is on designing system safety and cybersecurity into systems from the beginning of development. Another topic is how to infuse new technologies into complex systems over time. While the other fields in the department study how to create new types of propulsion, materials, human-computer interfaces, etc., Engineering Systems studies how to integrate these technologies into effective, useful, safe, and secure systems and how to operate these systems to ensure that the goals and constraints are maintained during their lifetime. We study these topics in collaboration with stakeholders from government, industry, and non-profit organizations.
System Architecture: System architecture is the study of the early-stage technical decisions that will determine the majority of the system’s performance. By identifying the most important initial technical decisions and exhaustively enumerating their options, the best potential designs are identified prior to the detailed design activities. The approach stands in contrast to a traditional trade-study perspective, where two to four points designs are compared, without reference to the intervening options or to a fully explored Tradespace. The emerging field of System Architecture aims to understand what patterns emerge across disparate domains, to gain an understanding of the making of good architecture. System architecture models look broadly across possible technologies, subsystems, and use contexts. Although each model employs problem-specific parameters, together they advance the state of the art by developing unique and generalizable approaches to structuring complex systems architecting problems that can be applied across disciplines.
Strategic Engineering: Strategic engineering involves the study of long-lived systems on Earth and in space. This includes the design and operation of critical infrastructures such as industrial manufacturing, transportation, earth observation, defense, water, energy, and food supply systems as well as the challenges of sustained human and robotic exploration and settlement of outer space. Research involves developing validated models and simulations to support strategic decisions under uncertainty, including a selection of technologies and system evolutionary pathways. Methodologies in strategic engineering include multidisciplinary design optimization, Monte Carlo simulation, multicommodity network flows, and other techniques based on discretizing systems both in time and space
System Safety and Security: The goal of safety and security engineering is to create new tools and processes to reduce losses, both in terms of human life and property, but also losses related to mission, scientific, and business goals. Engineering safer systems require multi-disciplinary and collaborative research based on sound system engineering principles, that is, it requires a holistic systems approach. A focus is on modeling and analysis tools based on systems theory to identify the constraints on how a system can achieve its goals and ensure the constraints are enforced while the goals are also achieved. The analysis includes hardware, software, humans, and organizational/management decision-making. Some current and recent research topics include creating concepts and requirements for defense systems, human-automation interaction, aircraft type certification, safety in airline operations, conceptual architectures for safety and cybersecurity, and safety of systems incorporating AI.
Educational Goals
The educational goal of the MIT graduate program in Engineering Systems is to provide students with a foundational understanding and a working knowledge of the technical issues surrounding the design, operation, and management of complex systems made up of a variety of hardware, software, human, and social components.
Successful graduates of the program will have:
- A fundamental understanding of system engineering in terms of the technologies, principles, methods and tools required to conceive, design, implement, and operate complex sociotechnical systems.
- The ability to take a holistic systems approach to problems that optimizes emergent properties of systems as a whole and can integrate independent technologies and system components to identify and achieve system goals and constraints.
- The ability to model and analyze complex, socio-technical systems with real world complexity and to influence their design and operation in the real world
- Generated research contributions to the current engineering systems body of knowledge.
Ever since the Wright Brothers (1903) managed to keep their flyer aloft, despite severe control challenges, it has been apparent that flying machines could not be designed without explicit consideration of human operators. Even recent unmanned areal vehicles (UAVs) require operators on the ground to program, remotely control, and monitor airborne operations from a distance. The study of human factors in aerospace covers the efficiency, safety, and comfort of pilots, passengers, and controllers. More recently, the safety, comfort, and effectiveness of astronauts have also been shown to be significantly impacted by factors such as equipment (space suits, tools), operating environment as well as established operating procedures. A thorough understanding of the interplay of these human factors is essential to ensure future mission success in the air and in space.
The air and space environments present significant challenges to the human body and mind. In particular, the acceleration, atmosphere, and radiation environments are of special concern to the well-being of pilots and astronauts. The complex interactions between humans and machines, including a high degree of automation in aerospace systems, is another major consideration for these complex sociotechnical systems. The study of humans in aerospace at MIT specializes in three areas:
Humans and Automation: Understanding cognitive workload and the degree to which automation (software, displays, controls, and algorithms) can assist humans in more effectively and efficiently operating and supervising aerospace vehicles while maintaining appropriate levels of workload, safety, and system awareness.
Physiology of Humans in Aerospace: Understanding how specific operating conditions in aerospace environments affect the health, comfort, and efficiency of human operators and passengers. This area encompasses the study of human visual perception, orientation, the effects of weightlessness and partial gravity on human physiology as well as specialized effects such as spatial disorientation. Applications include life support systems and crew protective devices, ranging from ejection seats to EVA suits.
Human Factors in System Design: This area of research includes the design of displays and controls for human interaction with complex systems, pilot and astronaut suits, and support equipment as well as the design of various aids for system status monitoring, crew coordination, communications.
Educational Goals
The overall educational goal of the MIT graduate program in Humans in Aerospace is to provide students with an understanding of the functioning of human psychology and physiology and the interactions with aerospace systems and environments. Students should develop a thorough understanding of the integration of humans in aerospace systems. This integration encompasses human-machine interactions and the nature of human error.
Successful graduates of the program will have achieved the following specific learning objectives:
- They will have acquired the ability to prescribe requirements and constraints on aerospace vehicles related to human comfort, effectiveness and safety.
- They will have gained an understanding of those aspects of human psychology and physiology which relate to ¬the design and operation of aerospace systems.
- They will be able to analyze aerospace accidents with a focus on human factors causes and contributing factors, and suggest design alternatives for both aviation and space systems.
- They will be able to directly contribute to the design of new space exploration vehicles, life support systems, and remote habitats, as well as to the redesign of existing systems.
- They will be able to design and evaluate cockpit and controller stations for advanced aircraft and for the control of mixed fleets of manned or unmanned vehicles.
- They will have exercised this knowledge for the design of a particular air or space mission in the context of a multi-disciplinary team.
Materials and structures are among the core disciplines in aerospace engineering, and many materials and structures advances have been borne out of the aerospace industry, e.g., carbon fibers and carbon fiber advanced composites. Study of Materials and Structures in Course XVI continues within the broad context of aerospace vehicle and systems design. Significant research themes include computational modeling of materials and structures, additive manufacturing (AM), fabrication and design of hybrid nano/micro materials, understanding and modeling of basic composite material and structural behavior/response, structural design, failure, and safety considerations, MEMS/NEMS materials and structures topics, and structural dynamics, among others. The graduate curriculum, and the broader research enterprise, benefits significantly from interactions with other Departments at the Institute including Mechanical Engineering (Course II), Materials Science and Engineering (Course III), Engineering Systems Division (ESD). Furthermore, Materials and Structures students have a long history of utilizing Harvard-MIT cross-registration to take advantage of numerous mechanics and materials courses offered at Harvard. Interactions with other Departments and institutions manifest in numerous ways, including rich research collaborations, co-advising of students, and cross-pollination of graduate courses.
educational goals
The educational goals of this field are to develop skills in the mechanics of materials and structures, including characterization, analysis, experimentation, and modeling of aerospace materials and structures under application-specific loading and environmental stimuli. Students often learn how to apply these insights in the study of new materials and/or structures with tailored behaviors that enable enhanced performance and the creation of new materials and their structures. Early coursework emphasizes foundational skills in analytical and computational solid and structural mechanics, which can be applied to a wide range of engineering challenges. Students then tailor their course selections to align with specific research interests, narrowing the focus to certain classes of materials (e.g., fiber-reinforced composites, monolithic high-temperature materials), structures (aerostructures, space structures, devices), environmental conditions (dynamics, elevated temperatures), fabrication and manufacturing approaches, or methods of analysis.
Space Propulsion comprises the propulsion technology required to reach space, as well as that which can be used to maneuver in space. The first category (space access) remains confined to chemical rocketry, although some attempts continue to be made at electromagnetic or other alternative means of high-g propulsion. The in-space category, by contrast, has evolved towards the use of more mass-effective types of thruster, such as Electric Propulsion rockets, solar sails, or electrodynamic tethers. The key difference, in this case, is the possibility of accumulating momentum for a long time, at the very low rate allowed by the limited supply of power available in space. Electric Propulsion (EP) has had a long laboratory maturation period, going back to the early 1960s, and has now reached the practical application stage, to the point that essentially all new satellites, commercial as well as scientific or military, now feature some form of EP as their nominal propulsion system. An intermediate category, Nuclear Thermal propulsion, exists in principle, although environmental, economical and sometimes political obstacles have prevented its application so far.
Chemical launch engines develop thrust levels that are many orders of magnitude larger than their in-space counterparts, and therefore any improvement to their performance would appear to offer correspondingly larger returns in terms of payload capability. On the other hand, for missions that require substantial cumulative velocity increments after reaching orbit, any improvement on the performance of the in-space propulsion system will reduce the payload mass for the launcher, thus allowing a proportional reduction of the launcher mass and cost. Chemical rockets have nearly reached their technological maturity, with improvements mainly being sought in the areas of reliability, cost, diagnostics, or controllability. Some exceptions are the potential for active control of combustion instabilities, or the possible miniaturization of these rockets. In contrast, Electric Propulsion and its extensions continue to offer many opportunities for incremental and even revolutionary developments. Aside from immediate utilitarian considerations, students that specialize in this field have an opportunity to learn and use physics concepts that go beyond the usual Aero/Astro range, including plasma physics, micro-and nano-technology, high-temperature fluid physics, etc, and can later access areas of technology that are also broader than usual.
Propulsion in general is a discipline that requires both, mastery of fundamentals and integration skills. A Space Propulsion specialist should be conversant with the requirements imposed by the overall mission goals (orbit dynamics, mass and power limitations, the relative cost of time, etc), those imposed by the spacecraft itself (thermal isolation between thrusters and bus, plume impingement effects and limitations, like voltage limitations and EM noise bounds), and methods for rationally selecting overall parameters (specific impulse, thrust and power level, etc) for reliable and sometimes optimal mission performance. But in addition, the specialist should also be familiar with the basic physics of the devices, which can be fairly sophisticated and unfamiliar to non- scientists. This is true even for engineers whose job will be to integrate propulsion systems into spacecraft, and it becomes essential for those whose job will be to develop and refine those thrusters. An exciting new direction in the development of Space Propulsion technology focuses on their application to small spacecraft, including the now popular backpack-sized CubeSats. Providing these small satellites with high-performance onboard propulsion would enable them to do not only what their bigger counterparts can do in earth orbit, but also explore deep-space destinations, including near-earth asteroids.
Some of the students selecting this field of study may choose to specialize in one of the fundamental disciplines that many in-space propulsion technologies depend on: Plasma Science. Plasma is defined as a collection of positively and negatively charged ions, negatively charged electrons, and neutral species. The main characteristic of the plasma is that, when looked at as a macroscopic average, it is electrically neutral, i.e., it has the same number of positive and negative charges. This neutrality eventually breaks down at the atomic scale, at the edges of the plasma, or at the interface with a wall, as well as for very rapid perturbations (faster than the so-called plasma frequency). Because of this, it is said that plasma is quasi-neutral. In a general sense, a plasma is like a multi-component fluid, and in many instances, its behavior can be described with the same set of tools used to describe regular fluids, with one exception: plasmas react to electric and magnetic fields, and as such, we need to consider stresses in addition to the hydrodynamic ones regular fluids experience. This response to electromagnetic fields enables the generation of propulsive forces that can be used for in-space applications. In addition, plasma chemistry is very rich and hence, in many cases, plasma has to be treated as a reacting flow, making plasmas extremely useful for a wide range of chemical applications such as combustion, fuel production, or CO2 conversion.
Aside from space-propulsion, in the past few years, new interests in Plasma Physics and integration of plasma technology into aerospace applications have emerged, Figure 2. Some of these applications include plasma-based flow control including boundary layer actuation and shock modification; and plasma-assisted combustion, or the unlocking of new reaction pathways through electron impact reactions to tackle challenging combustion regimes such as lean and ultra-lean combustion (for interest in low NOx emissions) or supersonic conditions. In addition, a deep command of plasma science and gas discharge physics is required to protect and mitigate against the natural environment in which aircraft and spacecraft live, e.g. protection of aircraft and launch vehicles against lightning strikes (plasma arcs).
A specialist in Aerospace Plasma Science and Technology should be well-versed in the fundamentals of plasma physics and be able to develop fundamental plasma models, computational techniques and algorithms as well as perform advanced experimental diagnostics, all of which are essential to advance the field. Given the interdisciplinarity of the field, the Aerospace Plasma specialist should also be familiar with the applications of interest, including aerodynamics, combustion, or aircraft design.
Educational Goals
The educational goal of the MIT graduate program in Space Propulsion Engineering is to provide students with a foundational understanding and a working knowledge of the many technical issues surrounding the design, operation and integration of the propulsion systems used in space. This includes both, chemical and non-chemical types of propulsion systems, although the latter category is emphasized for its closer ties to research conducted at MIT.
The educational goal of the MIT graduate program in Plasma Science and Technology is to provide students with a foundational understanding and a working knowledge of both the fundamentals of plasmas, ionized gases and electrical discharges as well as the many technological applications that they can enable, both existing and emerging.
Successful graduates of the program will have:
- Acquired an understanding of propulsion system requirements and constraints, as dictated by the overall space mission goals.
- Gained a fundamental familiarity with the physical principles that underlie the production and control of the high-energy gas, ions or plasma jets used in space propulsion.
- Acquired and understanding of the technical advantages of plasma technologies, including kinetic-assistance (plasma chemistry) and dynamics.
- Gained a fundamental familiarity with the principles of plasma science, including analytical and numerical modeling as well as experimental diagnostics.
- Generated research contributions to the current space propulsion engineering or aerospace plasma engineering body of knowledge.
Thousands of manned and unmanned spacecraft have been launched into space since the launch of Sputnik on October 4, 1957. We have landed men on the moon, visited every planet in our solar system with robotic probes, have established global telecommunication and navigation systems in Low Earth Orbit (LEO) and Geostationary Orbit (GEO), have launched numerous scientific and military satellites that gather a wealth of data about Earth’s climate, surface conditions, and weather. Despite all these accomplishments, many challenges remain:
Manned Space Exploration: A major driver in the coming two decades will be the return of human explorers to the Moon. It is becoming clear that a symbiosis of man-and- machine yields the best results in a challenging space environment. The optimal interactions between robots and humans during space operations need to be better understood. The responses of the human body to long-term weightlessness or sub-one-g conditions and relative isolation need to be researched and influenced as an enabler for long-distance human exploratory missions such as to the planet Mars, the natural extension of the Moon exploration program. A detailed understanding of interplanetary logistics, involving the flow of crews, consumables, and spares, among other supplies, needs to be developed to ensure the safety, effectiveness, and affordability of future operations.
Space Science: The rising awareness of climate change on Earth demands new sensor systems that can measure atmospheric and surface properties in real-time with full global coverage. This might be accomplished with small distributed arrays of spacecraft (distributed satellite systems) rather than traditional, large, and expensive single satellites.
The next generation of space observatories will tackle grand challenges such as the direct detection of Earth-like planets around neighboring stars, the investigation of the early universe after the Big Bang, the search for dark matter, and ultra-precise star maps among other investigations. These observatories will need to operate at far away Lagrange points such as the Earth-Sun-L2. They will be light-weight, cryogenic (<50K), and capable of precise micro-arc second pointing accuracy over several weeks of observing time. These requirements all represent daunting engineering challenges and important research opportunities.
Commercial Space: Beyond technical feasibility, further improvements are needed to make communications satellite systems more economical and better integrated into the global communications network including terrestrial fixed and cellular systems.
Space entrepreneurship is energized after the X-Prize in 2004 and numerous larger and smaller companies are now developing systems and concepts for commercial services in the area of space tourism (e.g. sub-orbital flights, orbital space hotels) as well as logistics services in near-Earth space (e.g. resupply of the International Space Station).
Military Space Systems: The modern military concepts of long-range all-weather, highly mobile warfare are enabled by use of space assets. Several shifts are taking place, where the goals are to provide “information superiority” to the individual warfighter as well as provide real-time, high bandwidth data connectivity between aircraft (manned and unmanned), spacecraft, ships and ground troops.
These challenges make it apparent that the success or failure of space systems depends not only on functionality and performance never before achieved by individual components but on the overall integration of the launch, space, and ground segments with human operators, in the context of policy, market, and technological uncertainty. This is the major challenge for space systems engineers.
In order to be successful as a space systems engineer a good working knowledge of satellite subsystems and general spacecraft technologies is required. One of the main tasks of systems engineers in practice is to conduct trades among alternative architectures and negotiate requirements with various stakeholders, including the customer, program management, and the various subsystem teams. The depth and advanced knowledge in at least one of the subsystem technologies (e.g. controls, structures, software and autonomy, payload engineering such as optics or radio frequency communications …) is paramount in order to establish acceptance, credibility, and fluency in the common technical vocabulary of space systems.
A second key aspect is teamwork and the communication necessary to make the team functional. No space system has ever been built by a single individual! While simple aircraft can be designed, built, and flown by a single person, this is not possible for satellites. The complexity of space systems and the requirements for launch, operations in the harsh environment of outer space, wireless communications around the globe make effective collaboration a key requirement. These two aspects, spacecraft engineering knowledge, and the development of a space system or mission as a team are at the core of our program in space systems.
One of the marks of excellence of MIT engineers is that they should both possess the breadth and technical depth at the same time. The space systems faculty deems it important space systems engineering remains strongly rooted in the technical disciplines. A candidate can tailor a program that provides depth in a technical specialty such as controls, structures and dynamics, software and autonomy, communications, astrodynamics, propulsion, and humans & automation among others while learning about most of the others. This approach to the curriculum design is described in the next section.
Educational Goals
The overall educational goal of our educational program in Space Systems Engineering is to provide students with a foundational understanding of the systems engineering principles, methods, and tools required to transform fundamental technical, economic, and societal requirements into an integrated space system solution.
This integration encompasses hardware, software, and humans, embedded in a clearly articulated value proposition and overall system architecture. Successful graduates of the program will have achieved the following objectives:
- They will have gained a fundamental understanding of space systems engineering in terms of the technologies, principles, methods and tools required to conceive, design, implement and operate a space system.
- They will have exercised this knowledge for the design of a particular space mission in the context of a development team.
- They will have established credibility and depth in a discipline with either a technology focus (e.g. control, optics, structures…) or process focus (e.g. optimization, manufacturing, space policy, operations…).
- They will have gained experience in the field by having applied this knowledge in the context of a space related industrial enterprise or government organization.
- They will have generated research contributions to the current space systems engineering body of knowledge.